Methanol to olefin, MTO, indirectly converts natural gas to a feedstock of the petrochemical industry by providing light olefins. MTO provides a new promising route to fill the increasing gap between supply and demand of propene through conversion of a non-oil resource
1
. The UOP/Hydro MTO process, the Lurgi process, and the DMTO (methanol or DME as feedstock) process are the major licensed available MTO technologies
2
-
5
, at the present time.
The first commercial scale installation of the UOP/Hydro MTO process was announced in 2011, and was planned to be in operation in 2013
6
. Honeywell UOP has its own MTO technology which now operates as Jiangsu Sailboat Petrochemical
7
with a production capacity of 833,000 metric tons per year as the largest single-train MTO unit in the world. The methanol feed was obtained from domestic coal.
Lurgi has specifically dedicated the methanol to propene, MTP, process with no ethylene product
2
. This company uses zeolite HZSM-5 as the catalyst to carry out this process. The key advantage of HZSM-5 is the longer lifetime of this zeolite against the SAPO-34 being used in MTO as a UOP’s proprietary catalyst
8
-
13
.
Researchers have focused on the improvement of coking and selectivity to light olefins over both catalysts
14
. Methanol converts to light olefin in an acid catalyzed route which is controlled by zeolite acidity and shape selectivity
15
-
19
,
9
,
20
-
22
. Particle size control and hierarchical pore structure affects the catalytic performance of zeolites in MTO reaction
23
,
14
,
24
. Metal incorporation in the zeolite structure is the one of routes to modify catalyst acidity with possible steric hindrance effects
25
-
30
. Experimental studies have proved
the positive effect of reducing the acidity level of the HZSM-5 zeolite on catalyst stability and selectivity to light Olefins
15
. The regions of strong acidic sites produce a rather large amount of aromatic compounds increasing the formation of carbon deposits in the pore structure of the zeolite
16
,
17
.
The incorporation of metal cations into the zeolite structure replaces protons of bridging hydroxyls, turning the Brønsted acid sites to Lewis ones. The substitution of aluminum for silicon in a silica covalent framework leads to a charge unbalance which must be compensated by extra framework cations, mainly alkaline. Alkali and alkali earth cationic zeolites are used mainly as adsorbent for industrial gas purification, e.g. CO2, to modify membrane matrix, and as ion exchangers for water softening. Basic property of alkali and alkali earth cationic zeolites renders these materials as potential basic catalysts
31
.
Cations possess determined locations in the pore structures of zeolites. For example, half of Na+ in Na-mordenite with a 12-membered elliptical Si−O rings are located in the middle of the small channels of compressed 8-membered rings in the site called (I) which is not supposed to be available to CO, CO2 and pyridine. The other half of Na+ ions are distributed between site IV, near the opening of the side pocket in the main channels made up by 12-membered rings and in the position VI, which is well inside the main channels near to five-membered side rings
31
. Different positions of cations or protons could result in different acidic strengths of these sites.
Olefins are produced on the acidic molecular sieves in MTO through a well-accepted hydrocarbon pool mechanism first proposed by Dahl and Kolboe
32
-
34
,
which means hydrocarbon intermediates after formation react with methanol leading to release of light olefins and other products from the intermediate species. Two cycles have been proposed on HZSM-5 in the MTO reaction
35
. In the olefin-based cycle, oligomerized olefins by methylation of C3+ alkenes are active intermediates
36
,
37
from which mainly olefin products form by cracking. This route is most favored on the acidic sites located over the channel walls in the pore structure of zeolites, producing predominantly
38
to propene and higher alkenes and significantly less carbonaceous deposits. The aromatic-based cycles, polymethylbenzenes and polycyclic aromatic as main intermediates
39
,
40
are methanol methylated and olefin released by elimination via side-chain and paring routes
41
,
42
. The aromatic-based cycle requires more space to form bulkier branched polycyclic intermediates. Therefore, acid sites located in the intersections of 2D sinusoidal and 1D straight channels, where cavities of 0.9 nm being formed, favors aromatic-based cycle which produces mainly
38
ethene, propene, and aromatics.
Taking into account above observations, redistribution of acid sites can regulate the dual-cycle hydrocarbon pool mechanism. Cation incorporation can be regarded as a synthesis strategy to tune the ratio of Lewis to Brønsted acid sites and redistribution of their locations.
It is also reported that concentration of Lewis and Brønsted acid sites on the zeolite can provide a synergistic effect on MTO product distribution through modification of hydrogen transfer rate.
The exchange of cations of alkaline earth and rare earth elements would decrease the overall acid strength of zeolites. The resulting Lewis acid sites, LAS, are generally weaker than the bridging Brønsted acid sites, BAS, in zeolites. This may decrease hydrogen transfer of exchanged zeolite which can diminish the cyclization and dehydrogenation, leading to less formation of alkanes and aromatic species
43
,
38
. Two routes have been suggested for the formation of alkane and aromatic species in MTO over HZSM-5 via hydrogen transfer (HT), i.e. methanol-induced HT and olefin-induced HT
44
. The former is affected by both BAS and LAS while the latter is driven by BAS
44
. Therefore, increase in LAS concentration induces a higher rate of the former route but does not promote HT reactions between olefins as reported for MTH conversion on HZSM-5. It should be noted that this conclusion was only obtained for Al-related LAS, and cannot be extended to other metal LAS formed by cation exchange
44
.
Moreover, the Aluminum coordination state in the zeolite framework could affect the MTO reaction. The synergy of extra framework Aluminum (EFAl) and Brønsted acid sites in zeolite catalysts was shown to change the reaction pathway, leading to significantly reducing the activation barrier of the initial C−C bond formation and accelerate the MTO reaction
45
.
Chemical modification of HZSM-5 by boron has been reported to increase the selectivity of propene by 48% and the lifetime of the catalyst by up to four times
16
. La and P incorporation to HZSM-5 (P-La-ZSM-5) increased catalyst lifetime from 126 hours to 188 hours, as well as the overall catalyst selectivity
25
. Modification of HZSM-5 by Iridium led to the 8% increase in the selectivity of propene as well as lifetime of the catalyst
26
. The performance of Fe-incorporated HZSM-5 indicated the increase in the selectivity of propene up to 6%
27
. The tuning of Si/Al in HZSM-5 by Fe isomorphous substitution exhibited a linear relation between the density of acid sites with selectivity to C2−C4 light olefins
28
. In a comprehensive study, Liu et al. modified the HZSM-5 catalyst with a Si/Al ratio of 220 by elements such as P, Ce, W, Fe, Mn, Gr, Mo, Ga, V and Ni. Their results showed that the selectivity to propene over P-HZSM-5 increased from 46.4% to more than 65.6%. The selectivity of propene after 3 h of reaction for different modifying elements has been reported as P > Ce > W > Mn > Fe > Gr > Mo > Ga > V > Ni
29
.
Al-Jarallah et al. reported that the Ca modified HZSM-5 exhibited the highest light olefin selectivity among various metals impregnated
30
. Results of Zhang et al. indicated that the performance of Ca-modified HZSM-5 in methanol to propylene reaction improved and the selectivity for propylene increased to 50%. This observation was explained by reducing the strong acid sites due to their interactions with Ca and the formation of Zeo-Ca-OH
46
,
47
. They also investigated the effect of Ca content on the performance of HZSM-5 with Si/Al ratio = 96 in the MTO conversion at methanol WHSV = 2.1 h−1. The size of catalyst particles was not reported. The optimum Ca content was determined = 6 wt.%, considering the aspects of stability (< 40 h) and light olefin’s selectivity (70−
80%). In another study of the effects of Ca incorporation to HZSM-5 structure with Si/Al = 40, samples of Calcium loaded-HZSM-5(Ca = 6 wt.%) prepared using different methods, were tested in MTO reaction at T = 500 °C and methanol WHSV = 8 h−1
48
. Suppression of aromatic
cycle in MTO reaction network by Ca incorporation, leading to longer life time (60 h) and higher selectivity to propylene (55%), was proposed. The superior catalytic activity of nano-sized particles of ZSM-5 zeolite was reported
24
. The positive effect of nano-sized ZSM-5 particles on the selectivity of propylene and catalyst life time in MTO reaction has been reported
23
,
14
. In this paper, the investigation of the effect of the incorporation of different content of calcium ions into nano-sized particles of HZSM-5 of high Si/Al (≈ 230) ratio with a hierarchical porous structure on the catalytic performance in MTO conversion is reported. In fact, the effect of Ca incorporation at the conditions of nano size of HZSM-5 particles and high Si/Al ratio favoring larger selectivity to light olefins and longer life time in MTO would be investigated.
1
Experimental
1.1
Material
Sodium aluminate (NaAlO2 > 98%) and tetrapropyl
ammonium hydroxide (TPAOH) [(C3H7)4NOH 1M solution] were purchased from Sigma Aldrich company.
Tetraethyl ortho silicate (TEOS) [Si(OC2H5)4 > 98%] was purchased from Acros company. Sodium Hydroxide
(NaOH > 98%), Ammonium nitrate (NH4NO3 > 99%) and synthesized calcium nitrate were prepared from the Merk company. Ethanol (C2H5OH > 96%) was obtained
from a domestic company.
1.2
Nanocatalyst preparation
To synthesize nano-sized ZSM-5 particles, the similar synthesis procedure reported elsewhere
49
was followed. In a typical synthesis, 34.4 mL of water was initially mixed with 0.014 g NaOH and stirred. 0.043 g of sodium aluminate (NaAlO2) was added to this solution. After complete dissolution of sodium aluminate in NaOH solution, 12.3 g of tetra-propyl ammonium hydroxide was added to the solution. After adding TPAOH, the solution was stirred at 25 °C for 2 h.
Then, the amount of 23.25 g of 96% ethanol was added to the solution and allowed to be well mixed with the initial solution. At this stage, the pH of the solution was 12.3. In the next step, the amount of 25.1 g of tetraethyl ortho silicate was added dropwise to the previous solution under vigorous stirring. The molar composition
of the resulting mixture was
1.44 TPAOH: 0.03 Al2O3∶13.8 SiO2∶222 H2O∶56.6 EtOH∶0.05 Na2O.
After stirring at 25 °C for 30 min, the gel mixture was refluxed without stirring at the temperature of the oil bath = 100 °C for 60 h. The solid white zeolite was separated by centrifuge, washed four times with DM water and dried in the oven at 105 °C overnight completely. The dried zeolite powder was calcined at 550 °C for 10 h with the ramp of 2.5 C°/min.
1.3
Ion exchange
The obtained Na-ZSM-5 zeolite was converted to the HZSM-5 by means of the ion exchange process. For this process, a solution of 1 molar ammonium nitrate was prepared and 20 mL of this solution was used per gram of catalyst. The catalyst suspension was stirred at 80 °C for 2 hours followed by centrifugal separation of solid product. This step was repeated three times, and then the drying step was carried out at 90 °C overnight. The dried powder catalyst was then calcined again at 550 °C for 6 h with the ramp of
2.5 C°/min.
1.4
Impregnation
Predetermined volumes of 0.1 M calcium nitrate solution were added to a specified amount of obtained solid product to adjust the composition of the final product to the Ca/Al ratios of 9, 27, 54. After stirring at 25 °C for 24 h, washing and centrifuge, the solid product dried at 90 °C overnight. The obtained powder was then calcined at 550 °C for 6 hours with the ramp of 2.5 C°/min.
1.5
Material characterization
1.5.1
Low angle X-ray diffraction measurement
Powder XRD spectra of synthesized nanocatalyst samples were recorded by the diffractometer Philips PW1730 that uses Cu K α1 radiation (λ = 1.5406 Å) with the power of 1200 watts (40 keV) to perform analysis with the 2θ scanning from 5−90° at the step size of 0.05°.
1.5.2
Surface area analysis
The surface areas of the catalysts were determined at −196 °C by surface analyzer micromeritics ASAP 2020. The specific surface area and porosity were calculated based on Brunauer-Emmett-Teller (BET) and t -plot models. The device measures the adsorption and desorption of nitrogen gas by the sample at a constant temperature of liquid nitrogen to determine the surface area and porosity. Prior to N2 adsorption-desorption process, the samples were degassed at 350 °C
for 4 h.
1.5.3
Field emission scanning electron microscopy (FESEM)
FESEM images and EDX spectrum were used to verify the formation, morphology and composition of nanoparticles. SEM images were obtained by TESCAN MIRA3 instrument with the EDX spectra recorded at the accelerating voltage of 15 kV and the beam current of 10 nA.
1.5.4
Fourier transformed infra-red
FTIR spectra of fresh and coked samples were recorded on a Testscan Shimadzu FTIR 8000 series spectrometer using the KBr pellet technique with the wavenumber range of 400−4000 cm−1.
IR absorption of pyridine adsorbed samples were taken on JASCO FT/IR-4600 type A, with standard light source detector TGS, Resolution of 4 cm−1, scanning speed of 2 mm/sec in 1300−1700 cm−1. Pyridine
adsorption was performed for 24 h after drying the sample at 120 °C overnight, kept away from air moisture
using silica gel prior to pyridine adsorption. A home-built system was used for simultaneous contacting four isolated samples with pyridine vapor in argon for pyridine adsorption. Samples were kept in silica gel desiccator before IR absorption test.
1.5.5
Temperature programmed desorption (NH3 TPD)
The temperature programmed desorption analysis was performed by using reaction setup connected to the required gas supplies and a TCD detector. In a typical TPD analysis, an amount of 0.10 g of calcined sample packed in a quartz U-tube between quartz particles,
0.2 g in each side, was degassed by heating up to 250 °C
at the temperature ramp of 10 °C/min and hold time of 1 h, followed by cooling to 100 °C in 2 h under argon flow of 30 mL/min. The absorbing basic Ammonia mixture gas stream (5% NH3 in He) was introduced to the sample at the flow rate of 40 mL/min for 15 min. Then, the sample was purged under Helium flow of
30 mL/min for 50 min. During purge time, the TCD detector was turned on and the baseline of the detector signal was stabilized. Helium flow rate was turned to 30 mL/min and the sample was heated to 700 with the rate of 10 °C/min. The desorption profile was obtained from the TCD signal recorded during the heating period.
1.5.6
Thermogravimetric analysis (TGA)
About 5 mg of the prepared zeolites were loaded in a TA Instruments SDT -Q600 to measure TGA profiles. A stream of Air was introduced to the zeolite sample being heated up to 650 °C with the temperature ramp of 10 °C/min.
1.6
Reaction setup
In the experimental reaction set-up, catalytic reaction was carried out inside a quartz u-shaped tube reactor placed in an electric furnace, connected to gas chromatograph at the outlet for online product analysis. In each experiment 0.2 g of catalyst particles of size 1−
1.5 mm, were placed in a quartz U-tube with inner diameter of 5 mm and then the catalyst bed was heated up to 490 °C for 1 h under Argon flow = 20 mL/min controlled by a MFC. A bottle of liquid methanol stirred at 650 rpm and 45 °C was bubbled via an Argon stream of 20 mL/min at the atmospheric pressure to obtain the methanol WHSV = 5.55 h−1 in the catalyst bed. The mixed feed stream was directed to the quartz U-tube at 490 °C through a heated line and was allowed to contact the catalyst bed for at least 2 h. Afterwards, the reactor test was continued for each catalysts at the lower temperatures of 440 and 390 °C. Finally, the furnace was reheated to 490 °C and argon flow reduced to 10 ml/min in order to compare the performance of the catalyst sample at the methanol WHSV = 3.27 h−1. Product flowed out of a quartz U-shape reactor passing through a heated line after which the product stream was condensed at room temperature. The weight and density of the liquid product was measured. The density and GC analysis showed it mainly composed of unconverted methanol and water.
Long term performance tests were carried out on the 0.1 g of catalyst particles with a methanol bubbler using argon flow rate = 20 mL/min at the methanol WHSV of ca. 7.5 h−1. Gas products were analyzed online by Gas Chromatograph (Agilent 7890 A) equipped with a FID detector and HP-Plot Q capillary column (30 m × 0.53 mm × 40 μm) under He carrier flow. In each GC analysis of product samples, the oven temperature was held constant at
60 °C
. The temperatures of injection inlet and FID detector were set to 250 and 300 °C, respectively.
2
Results and discussion
2.1
XRD results
The XRD patterns of the prepared samples were presented in
Figure 1
. All diffraction peaks appeared in the expected 2θ degree confirming the formation of MFI-structure of ZSM-5 for all sample powders. Calcium added to the structure of ZSM-5 did not form a nano cluster on the external surface of zeolite particles as there was no relevant impurity reflection in the XRD patterns. Moreover, as shown in
Figure 1
, the intensity of the diffraction peaks of modified catalysts was reduced, which may indicate the incorporation of metals into the microporous structure of zeolites.
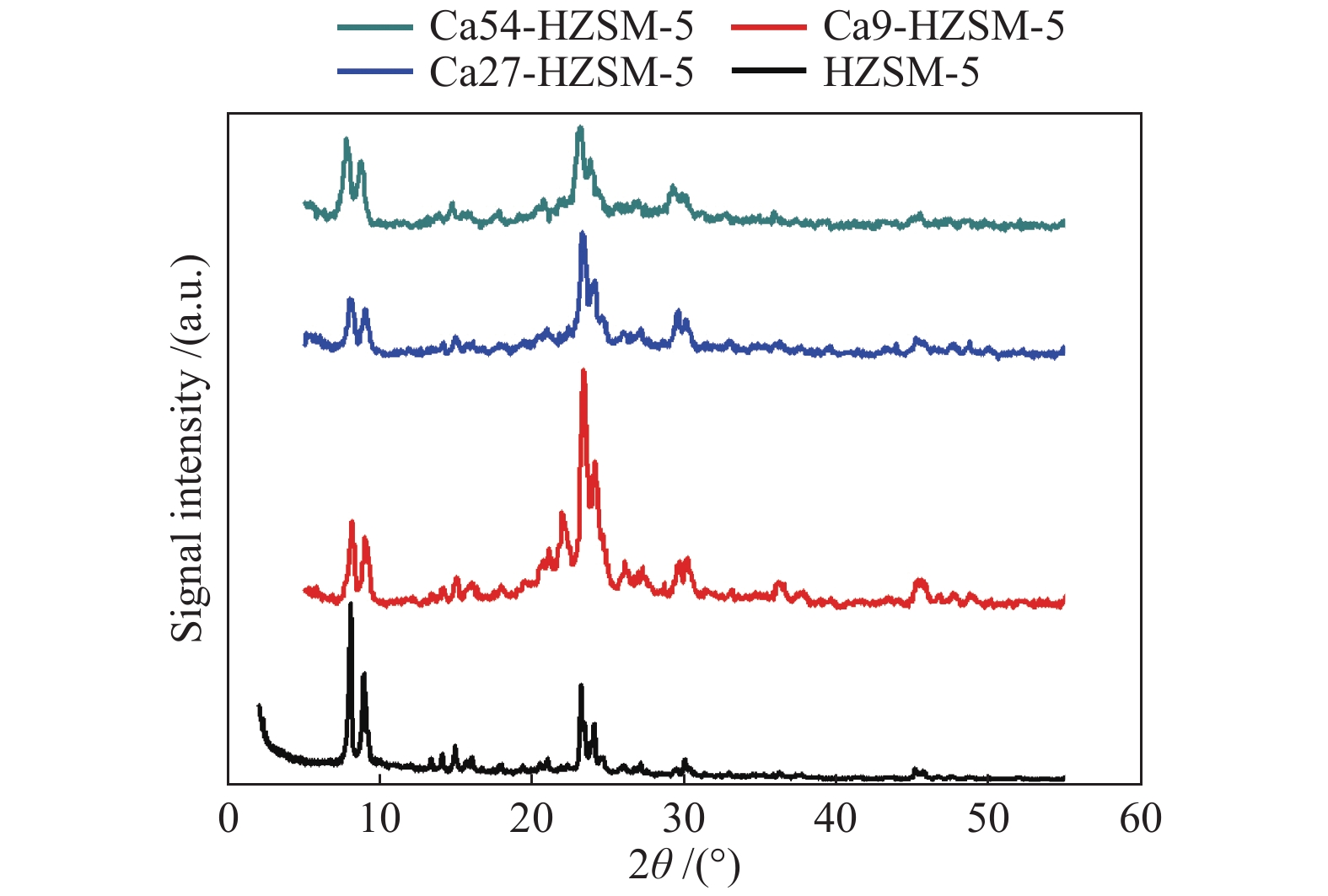
Fig. 1
XRD patterns of the prepared samples
2.2
Surface Analysis
N2 adsorption desorption isotherms of the prepared catalysts were given in
Figure 2
. The sharp increase of adsorption of N2 over the samples at the low p /p 0 confirmed the formation of a microporous zeolite material. Samples exhibited a type-IV isotherm with two hysteresis loops at relative pressures higher than p /p 0 ≥ 0.3 and 0.5, indicating existence of mesopores. These hysteresis loops (H3 type) are usually associated with capillary condensation in mesopores.
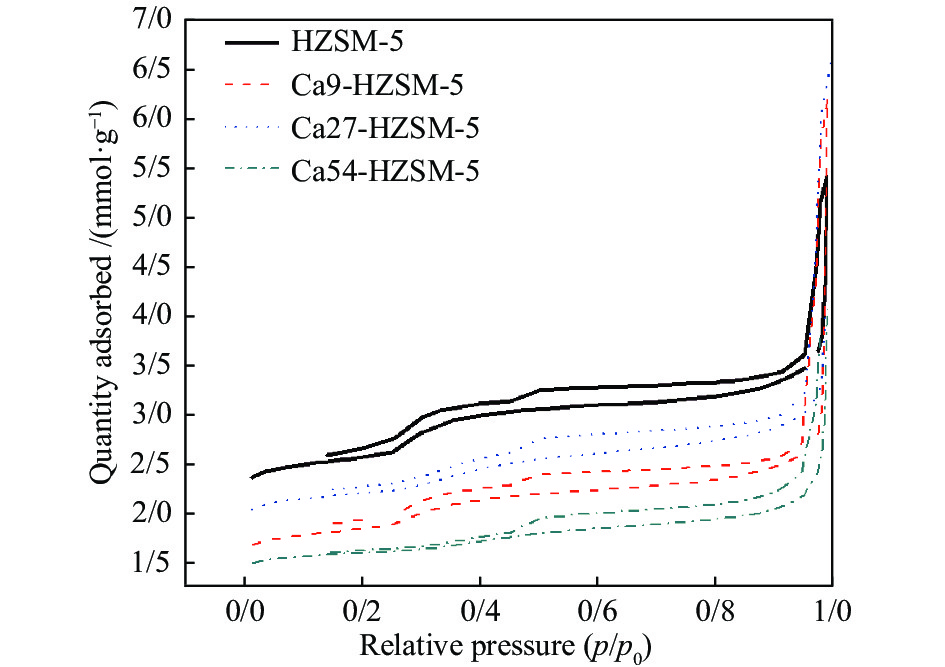
Fig. 2
N2 adsorption/desorption isotherms of the prepared catalysts
The micropore size distribution plot obtained via t -plot method using HJ model was given in Figure S1(a) of the supplementary material file. The parent HZSM-5 showed the largest adsorption capacity. Ca27-HZSM-5 and Ca54-HZSM-5 samples presented the highest and the lowest surface area among the calcium modified zeolites, respectively.
In
Table 1
, the results of pore size and specific surface areas of the synthesized samples were presented. It is observed that by the incorporation of metal species to the catalysts, the specific surface areas of the catalysts were reduced. This result indicated that the pores volume has been occupied by the metal atoms with the incorporation of the metals, at least to some order. The micropore volume of Ca9-HZSM-5 and Ca54-HZSM-5 decreased to about 0.05 from
0.07 (cm3/g) in the parent HZSM-5 as a result of metals incorporation inside their micropore volume of crystalline structure. Ca27-HZSM-5 presented more micropore volume respect to the other two Ca incorporated HZSM-5 zeolite.
According to the data in
Table 1
, the size of micropores of maximum distribution in the parent HZSM-5 was 5 Å, which increased with Ca incorporation
as the corresponding pore sizes increases to 5.2 and
5.5 Å in Ca27-HZSM-5 and Ca54-HZSM-5 catalyst, respectively. The micropore size enlargement is a result of BET model to fit the reduced pore volume measured.
Tab.1
Pore structure properties of the prepared catalysts obtained from N2 Adsorptive isotherm
|
|
|
|
|
|
|
|
|
0.129−0.071
|
193
|
152
|
42
|
2.6
|
11
|
0.50
|
|
0.097−0.049
|
139
|
105
|
34
|
2.8
|
11
|
0.50
|
|
0.112−0.065
|
165
|
139
|
27
|
2.8
|
11
|
0.52
|
|
0.083−0.048
|
120
|
103
|
17
|
3.2
|
11
|
0.55
|
Two flat and wide hysteresis loops
50
can be observed in the N2 isotherms of
Figure 2
can be attributed to slit mesopores pores or ink-bottle-shaped mesopores with small openings, or it can be due to the effect of a pore network. These mesopores, pore size distribution shown in Figure S1(b) probably were formed due to the silicon dissolution in the course of synthesis of the parent HZSM-5. Two mesopore sizes, given in
Table 1
, were measured for each sample. The larger mesopore of samples provides the insignificant mesopore volume respect to the smaller pore size. The calculated N2 adsorption capacity of the metal-modified HZSM-5 catalysts were less than that of the parent HZSM-5, probably due to the reduced crystalline order in the samples, cage size, and/or pore blockage as a result of metal incorporation.
2.3
The SEM Analysis
The SEM images of catalyst powders were shown in Figure S2. As it can be observed in the images, the crystal particles are interconnected agglomerates of nano (sub-micron) size in the range of 100−200 nm with nearly spherical shape. No distinct difference in the morphological properties of particles of the prepared samples can be observed. Hence, Ca impregnation
didn’t
lead to distinct morphological effects on the HZSM-5 particles. It is a desirable feature to compare the MTO performance of the prepared samples without the concern of probable effects of physical properties of samples particles such as their size or shape on their catalytic performances.
In
Table 2
, composition data of the synthesized samples obtained by EDX spectroscopy were given. According to the results, Al content of samples was less than 1 wt% as the target value of Si/Al in the synthesis procedure is as large as 230. Despite the low accuracy of EDX results for low content element in the samples, i.e. calcium content, the expected increasing trend of Ca content values of samples was followed. The calculated Ca/Al ratios for CaX-HZSM-5 (X = 9, 27, 54), given in
Table 2
, equal 5.7, 19, and 34, respectively.
Tab.2
Elemental composition of the prepared catalysts obtained from EDX spectra
|
|
|||
|
|
|
|
|
|
68.66
|
72.48
|
71.25
|
71.61
|
|
0.10
|
0.20
|
0.12
|
0.19
|
|
31.18
|
26.19
|
26.35
|
21.68
|
|
−
|
1.14
|
2.27
|
6.52
|
|
312
|
131
|
220
|
114
|
|
−
|
|
|
|
2.4
FT-IR results
FT-IR spectra of the prepared catalysts were recorded in the range of 400−4000 cm−1, as shown in Figure S3. The band around 450 cm-1 represents the Si−O−Si bending vibration of the five-membered rings in the zeolite framework
51
. The absorption band about 550 cm−1 was assigned to the external symmetric stretching vibration of the five-membered rings in MFI zeolites
52
,
53
,
51
,
54
.
The absorptions band at 800 cm−1 and 1100 cm−1 appeared due to the asymmetric stretching of external and internal linkages, respectively
53
,
52
.
The peak at 875 cm−1, appeared and intensified with the Ca content in the modified zeolites, could be attributed to the Ca−O vibrations. It could be considered as an indication of Ca incorporation in the structure of HZSM-5.
An external asymmetric stretching vibration at 1225 cm−1 originates from the vibrations of structures containing four chains of 5-membered rings
52
,
51
,
55
. It can be summarized that the absorption bands around 790−1440 cm−1 (asymmetric stretching vibration of the T−O−T modes) appeared in all samples, due to internal linkage in SiO4 or AlO4 of zeolite lattice
16
.
The trace amount of unburnt template or hydrocarbon impurities could be detected by the C−H stretching absorption bands at 2840−2940 cm−1
56
-
58
.
FT-IR spectra in the range of 3500−3800 cm−1 exhibit vibration of surface hydroxyl (OH) groups. The bands at 3610 cm−1 and 3680 cm−1 were attributed to the vibration of bridging Si−OH−Al and extra-framework Al−OH groups, respectively
52
,
59
-
61
. Bridging OH in Si−OH−Al is considered as the strong Brønsted acid sites of zeolites. The band at 3740 cm−1 assigned to the lattice terminal Si−OH groups
59
,
60
,
62
,
61
.
Yarulina et.al. calculated that OH stretching frequency of HZSM- 5 at 3684 cm−1 shifted upwards to 3848 cm−1 and 3796 cm−1 for the straight and curled Ca chains (CaOCaOH+) in the Ca-ZSM-5 catalyst
48
. Therefore, the absorption at 3840 cm−1 could be assigned to stretching vibration of straight Ca chain in the Ca-exchanged HZSM-5 catalysts.
To evaluate the distribution of BAS and LAS in the synthesized zeolites, samples are first dried in
120 °C and then treated with pyridine vapor at room temperature for 24 h. Samples were stored in the dry Argon atmosphere prior to analysis. According to the literature, the lone pare orbital of adsorbed pyridine would interact with Lewis acid sites absorbing IR at 1456 and 1510 cm−1, which BAS shift this absorption band to higher IR frequencies, i.e. 1545 and 1645 cm−1, respectively
63
. Thus, as it is common, two bands at 1456 and 1545 were selected to calculate LAS and BAS percentage in the prepared zeolites. Two broad peaks were clearly detected in 1700−1300 cm−1 from which the band in 1580−1380 cm−1 was chosen for calculation of acid site distribution. This vibration band in FT-IR spectra of pyridine adsorbed samples are plotted in the supplementary material file as Figure S4 as well as the deconvoluted peaks. Bands at 1456 and 1545 were plotted with thick dashed lines to be recognized from other bands. The remaining bands would be related to other vibrations of chemically or physically adsorbed pyridine.
The center of deconvoluted peaks and their area, the calculated BAS/LAS ratio and LAS percentage were reported in
Table 3
. BAS vibration peak of Ca9-HZSM-5 showed a shift to lower wavenumbers of pyridine adsorbed on BAS (1547 => 1540 cm-1) with respect to other catalysts, indicating weaker interaction of adsorbed pyridine with BAS in Ca9-HZSM-5. It could be considered as a decline in the BAS strength of Ca treated HZSM-5 at Ca/Al = 9. No significant shift of vibration wavenumber of adsorbed pyridine on Lewis acid sites of the prepared catalysts was observed. At Ca/Al = 9, the BAS site of zeolite constitutes the major part (≈ 70%) of acid sites while the LAS are in the majority (≥ 78%) in the prepared zeolites at Ca/
Al ≥ 27. The decline of strength of Brønsted acid sites up to a Ca content followed by their strength enhancement with further Ca incorporation could be assumed. It implies that BAS strength is dependent on the concentration of near LAS sites. It seems that high concentration of LAS leads to loss reducing effects on BAS strength, which may be caused by directional removal or strengthening of polar forces. Such a trend was exactly observed in TPD data, explained in the next section.
Tab.3
Center, area of IR BAS and LAS peaks of IR spectra of pyridine-adsorbed catalysts and their distribution
|
|
|
|
|
|
*calculated by taking the ratio of molar absorption IR coefficients εLAS/εBAS = 1.5
|
|||||
|
BAS
|
1549
|
0.1608
|
22.7
|
4.2
|
LAS
|
1454
|
0.0109
|
|||
|
BAS
|
1540
|
0.1248
|
2.16
|
31.6
|
LAS
|
1456
|
0.0889
|
|||
|
BAS
|
1547
|
0.1632
|
0.276
|
78.3
|
LAS
|
1457
|
0.9101
|
|||
|
BAS
|
1546
|
0.0253
|
0.222
|
81.8
|
LAS
|
1456
|
0.1754
|
The calculated percentage of LAS in the prepared catalysts show a direct relation between Ca/Al ratio of synthesis and their calculated Lewis acid site percentage. It is noteworthy that the variation of acid site distribution nearly loses its dependence on Ca content after Ca/Al = 27. EDX data confirmed that Ca content of Ca54-HZSM-5 is near to 3 times of Ca27-HZSM-5. A larger increase of LAS percentage in Ca54-HZSM-5 with respect to Ca27-HZSM-5 is expected. It seems that steric limitations imposed by Ca cations on pyridine molecules prohibited their diffusion to Lewis acid sites placed in the most inner parts of catalyst particles.
2.5
Ammonia TPD Results
The acidic properties of the Ca incorporated HZSM-5 catalysts as well as the parent HZSM-5 catalyst were studied by NH3-TPD technique and the obtained desorption profiles were displayed in
Figure 3
.
The NH3-TPD profiles were deconvoluted to three or four NH3 desorption peaks, including the first peak around 160 °C, the second one in the range of 358−
426 °C, and the third one in the range of 408−575 °C. The first resolved peak was related to the physisorbed NH3. The second peak was related to terminal −Al−OH and −Si−OH and −Ca−OH as weak Brønsted acid sites. The last resolved peak around 500 °C was assigned to strong Brønsted bridging OH (Al−OH−Si) or Ca related Lewis acid sites. The area of the last two peaks of Ca incorporated zeolites strongly increased. Therefore,
the Ca associated adsorbed ammonia must account for a significant portion. Brønsted acid sites convert to Lewis acid sites with incorporation of Ca cations in HZSM-5 structure, as previously reported
48
.
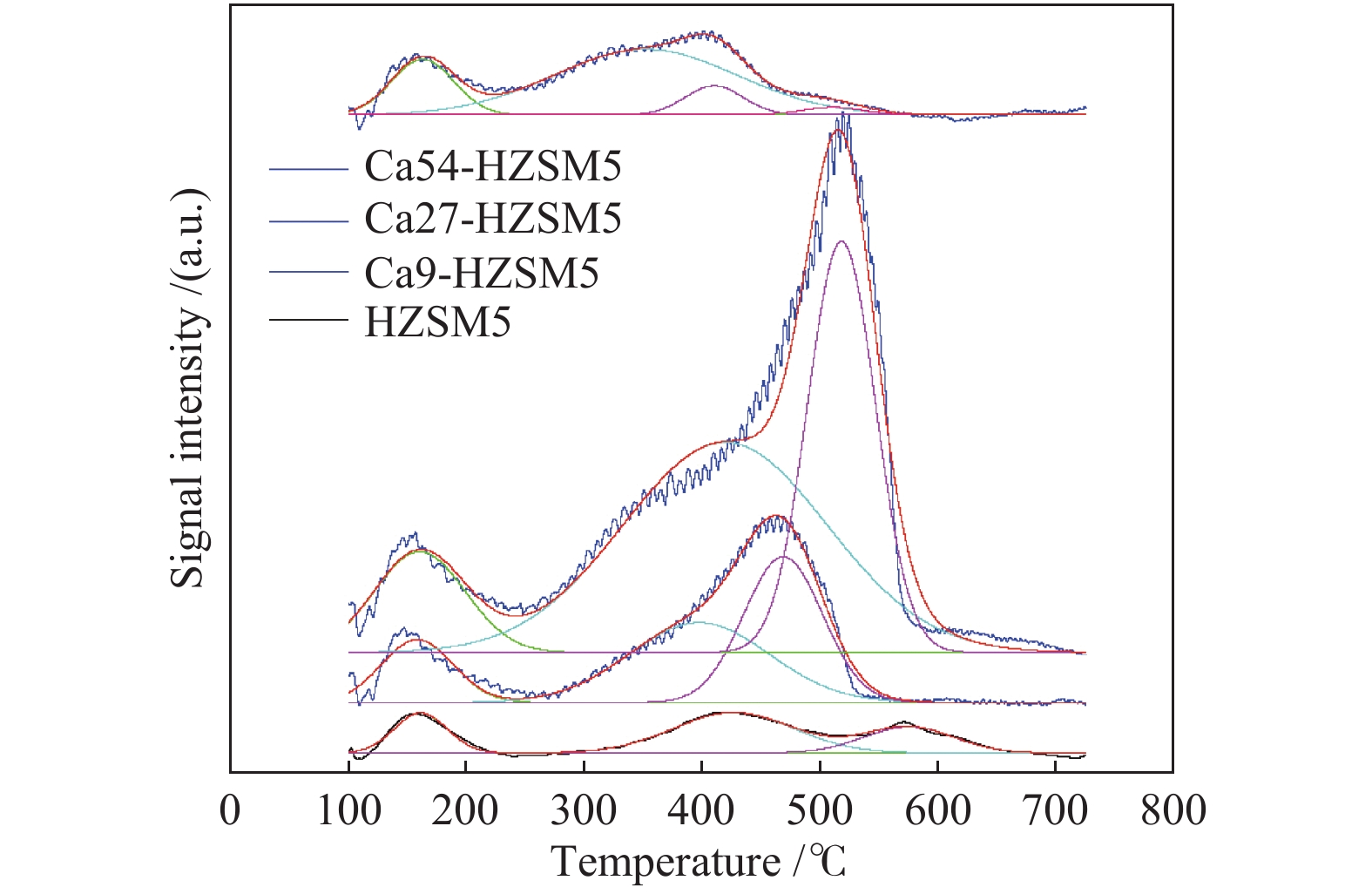
Fig. 3
NH3-TPD profiles of HZSM-5 and Ca incorporated HZSM-5 zeolites
Maximum temperature, area and height of deconvoluted peaks in the NH3-TPD profiles of the prepared catalysts were presented in
Table 4
. The height of peaks was used for comparing the density of corresponding acid sites because the resolved peaks are broad and overlapped.
Tab.4
Maximum temperature, area and height of deconvoluted peaks in the NH3-TPD profiles of the prepared catalysts
|
|
|
|||
|
Centerline Temp., °C
|
161
|
426
|
575
|
|
Area
|
1177
|
2721
|
1316
|
||
Height
|
21
|
21
|
13.8
|
||
|
Centerline Temp., °C
|
158
|
397
|
468
|
|
Area
|
2515
|
6263
|
6440
|
||
Height
|
33
|
42
|
77
|
||
|
Centerline Temp., °C
|
160
|
419
|
518
|
|
Area
|
5145
|
24037
|
15611
|
||
Height
|
53
|
110
|
217
|
||
|
Centerline Temp., °C
|
162
|
358
|
408
|
518
|
Area
|
1878
|
7255
|
675
|
low
|
|
Height
|
29
|
35
|
13
|
low
|
According to the data in
Table 4
, Si−OH, Al−OH (small share due to low Al concentration), Ca−OH acid sites (2th peak) were appeared in the TPD profile as a peak with the center around 358−426 °C with the following order of height size in the prepared zeolites:
Ca27-HZSM-5 > Ca54-HZSM-5 > Ca27-HZSM-
5 > HZSM-5
Si−OH−Al and Lewis acid sites (3th peak) were appeared in the TPD profile as a peak of centerline at about 408−575 °C with the following order of height size
Ca27-HZSM-5 > Ca9-HZSM-5 > HZSM-5 > Ca54-
HZSM-5
Although, the height and area of the second and third peaks varied considerably, it cannot be related directly to the density of weak or strong acid sites. Theoretically, the Ca cation incorporated should replace two protons meaning that a LAS replaces two BAS. Hence, a decrease of total density of acid sites should be expected. However, the TPD profiles of Ca incorporated zeolites showed increased adsorbed ammonia. One explanation could be the possibility of multiple adsorption of ammonia on Ca Lewis acid sites rather than single adsorption by OH group.
FT-IR spectra of pyridine adsorbed samples indicated decrease of BAS strength along with no change of LAS strength. This observation may explain the initial shift of the third TPD peak to lower temperature at Ca/Al = 9 followed by strength recovery of the corresponding acid sites at Ca/Al = 27. Considering the larger portion of LAS in Ca27-HZSM-5, the effect of weakened BAS on peak shift decreases with Ca content. Hence, the third peak shifts back to higher temperature, though still lower than that of the parent zeolite.
The weak Brønsted acid sites accounted for the 2nd NH3 desorption peak originated from terminal OH, i.e. Al−OH, Si−OH, or Ca−OH, respectively.
The 3th peak in the NH3-TPD spectra was assigned to the adsorption of NH3 onto strong acid sites resulting from bridging OH (Si−OH−Al), extra-framework Al and Ca as Lewis acid sites.
It is obvious from
Figure 3
that Ca incorporation in Ca9-HZSM-5 and Ca27-HZSM-5 has resulted in at least two different Ca related acid sites participating in ammonia adsorption at different strengths. It is noteworthy that intensification of the second and third peaks accompanied with a downward shift of their maximum temperature, showing decline of the relevant acid strength.
Ammonia TPD data can be summarized as follows. The second Peak in TPD profiles grows in Ca/Al ratios of 9 and 27 due to increase of Ca−OH while its centerline shifts to lower temperatures with respect to that of HZSM-5 catalyst indicating less Brønsted acid strength of Ca−OH than Si−OH or Al−OH.
The third peak includes both bringing OH sites and LAS. Its area grows for samples of Ca/Al ratios = 9 and 27 as its centerline shifts to lower temperatures at Ca/Al ratio = 9 and shifts back to higher temperature again at Ca/Al = 27. FT-IR data of pyridine adsorbed samples confirmed such a variation of strength in BAS sites. Considering the significant portion of Ca LAS in the third peak in the Ca incorporated zeolites, the strength of their LAS should be less than original BAS in the parent HZM-5. Both LAS and BAS are almost inaccessible to ammonia in TPD analysis of Ca54-HZSM-5 catalyst. It seems −Ca−OH, mostly on the external surface (peak center at 358 °C), has remained accessible in the TPD experiment on Ca54-HZSM-5.
2.6
MTO Performance tests
Table 5
shows the results of catalytic performance including the calculated values of methanol conversion, selectivity and yield of ethylene and propylene for the prepared catalysts at the different operating conditions. The MTO performance data of the prepared catalysts are also shown in
Figures 4
−
5
and Figure S5 in the supplementary material file. As expected, the highest methanol conversions were observed in the reaction at the highest temperature, i.e. 490 °C, and the lowest methanol WHSV, i.e. 3.3−4.0 1/h.
It can be observed from data in
Table 5
which are also plotted in
Figure 4
−
5
and Figure S5, methanol conversion increases with temperature for all catalyst samples. At the higher temperature, methanol converts faster to products leading to the higher conversions for the prepared catalysts. According to
Figure 4 (a
,
b)
, the selectivity to ethylene and propylene samples increases with temperature over all catalysts. The yield of product species (Yi ), the ratio of molar flow rate of product species (Fi ) per molar flow rate of methanol feed (Fmethanol,in), calculated by the multiply of methanol conversion and selectivity of species (Si = ni Fi /
(Fmethanol,in- Fmethanol,out)), i.e. Yi = Fi /Fmethanol,in; provides a certain comparative performance variable among a set of catalysts. ni denotes the number of carbon atoms in one molecule of species i. According to data plotted in
Figure 5 (a
,
b)
, the yield of both ethylene and propylene
enhances with temperature.
The decrease of space velocity of feed stream in the reactor resulted in the increase of the yields of ethylene (
\( {\rm{C}}^{=}_{2} \)
) and propylene (
\( {\rm{C}}^{=}_{3} \)
) at 490 °C for all catalysts as the increased residence time converts more methanol to light olefins.
The largest yield of propylene over the prepared catalysts, as can be observed in
Figure 5
, was achieved by Ca27-HZSM-5 at the studied operating conditions, especially at lower temperatures. The yield of
\( {\rm{C}}^{=}_{2} \)
and
\( {\rm{C}}^{=}_{3} \)
with the Ca27-HZSM-5 catalyst was 0.11 and 0.14 at 490 °C and WHSV = 5.5 h−1, respectively. The Ca27-
HZSM-5 provided the highest
\( {\rm{C}}^{=}_{2}+{\rm{C}}^{=}_{3} \)
yield among all the prepared catalysts, especially at lower WHSV of methanol, most probably due to the increased portion of Ca LAS with less acid strength than BAS.
Tab.5
methanol conversion, selectivity and yield of ethylene, propylene and C2−C4 olefins obtained by the prepared catalysts at the different reaction temperatures and space velocities of methanol feed stream
Catalyst
|
Temp., °C
|
WHSV,
1/h
|
Conversion
|
Ethylene
Selectivity (Yield)
|
Propylene
Selectivity (Yield)
|
Select- ivity to
\( {\bf{C}^{=}_{2}+{{C}}^{=}_{3}} \)
|
Yield of
\( {\bf{C}^{=}_{2}+{{C}}^{=}_{3}} \)
|
\( {\bf{C}^{=}_{3}/{{C}}^{=}_{2} }\)
Ratio
|
|
490
|
5.55
|
0.94
|
0.26 (0.12)
|
0.45 (0.14)
|
0.71
|
0.26
|
|
440
|
5.55
|
0.87
|
0.22 (0.10)
|
0.42 (0.12)
|
0.64
|
0.22
|
|
|
390
|
5.55
|
0.75
|
0.20 (0.08)
|
0.39 (0.10)
|
0.59
|
0.18
|
|
|
490
|
3.27
|
0.96
|
0.28 (0.13)
|
0.48 (0.15)
|
0.76
|
0.28
|
|
|
|
490
|
5.55
|
0.91
|
0.26 (0.12)
|
0.44 (0.13)
|
0.70
|
0.25
|
|
440
|
5.55
|
0.85
|
0.23 (0.10)
|
0.41 (0.12)
|
0.64
|
0.22
|
|
|
390
|
5.55
|
0.71
|
0.21 (0.07)
|
0.39 (0.09)
|
0.60
|
0.16
|
|
|
490
|
3.27
|
0.93
|
0.32 (0.15)
|
0.51 (0.16)
|
0.83
|
0.31
|
|
|
|
490
|
4.5
|
0.85
|
0.26 (0.11)
|
0.51 (0.14)
|
0.77
|
0.25
|
|
440
|
4.8
|
0.84
|
0.24 (0.10)
|
0.48 (0.13)
|
0.72
|
0.23
|
|
|
390
|
5.0
|
0.85
|
0.24 (0.10)
|
0.46 (0.13)
|
0.70
|
0.23
|
|
|
490
|
3.96
|
0.97
|
0.30 (0.14)
|
0.57 (0.18)
|
0.87
|
0.32
|
|
|
|
490
|
6.10
|
0.66
|
0.27 (0.09)
|
0.55 (0.12)
|
0.82
|
0.21
|
|
440
|
5.88
|
0.72
|
0.25 (0.09)
|
0.52 (0.12)
|
0.77
|
0.21
|
|
|
390
|
5.00
|
0.66
|
0.22 (0.07)
|
0.50 (0.11)
|
0.72
|
0.18
|
|
|
490
|
3.60
|
0.77
|
0.34 (0.13)
|
0.66 (0.17)
|
1.00
|
0.30
|
|
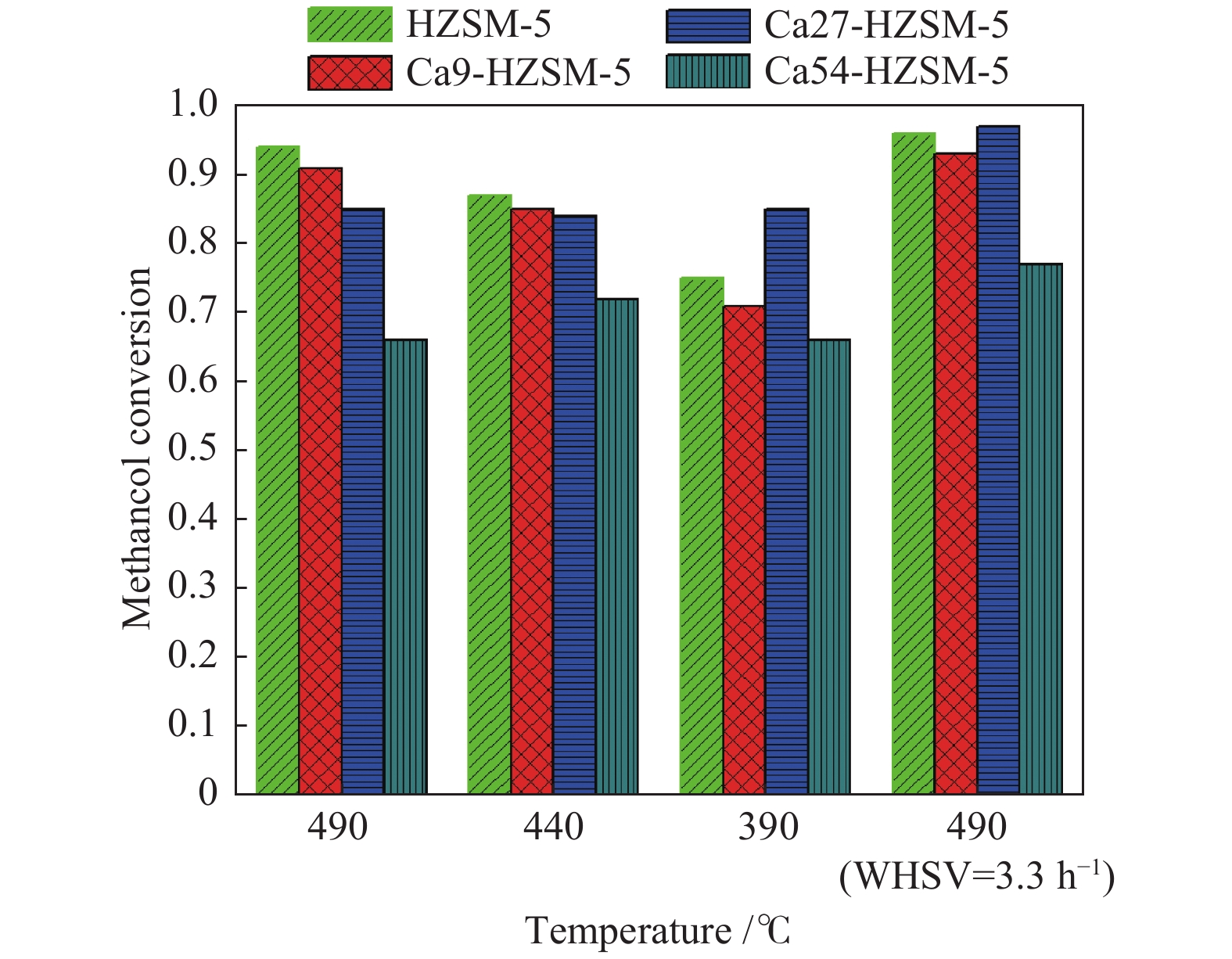
Fig. 4
methanol conversion versus temperature for the prepared catalysts in the MTO conversion, Pressure = 100 kPa, methanol WHSV = 8 and 4 h−1, Argon flow = 20 SCCM
Ca54-HZSM-5 with the lowest methanol conversion, is the most selective catalyst toward propylene meaning that less conversion of methanol favors propylene production. The TPD profile of Ca54-HZSM-5 showed reduced ammonia adsorption which was related to the diffusion barrier induced by populated Ca cations inside pore structure. The highest LAS content with limited accessibility led to the lowest conversion with highest propylene selectivity.
Comparison of methanol conversion on the prepared catalysts indicated that HZSM-5 catalyst showed the highest conversion, i.e. Ca incorporation into HZSM-5 led to the loss of MTO activity. However,
the parent HZSM-5 presented the smallest selectivity to
\( {\rm{C}}^{=}_{2}+{\rm{C}}^{=}_{3} \)
Obtained. Therefore, it could be concluded that the incorporation of Ca in the structure of HZSM-5 led to the reduction of conversion rate of methanol along with the increased selectivity to
\( {\rm{C}}^{=}_{2}+{\rm{C}}^{=}_{3} \)
in the light olefin products.
According to a relevant study
48
, Ca incorporation into HZSM-5 with Si/Al = 46 (1 < Ca/Al < 6) improved
the selectivity to propylene in MTO reaction in the expense of ethylene selectivity. In this study, the decline of strength of acid sites and suppression of hydride transfer and oligomerization reactions on significantly weaker acid sites were suggested as the reasons for the effect of Ca incorporation, i.e. diminishing aromatic cycle and lowering ethylene selectivity. However, our data did not show a trade-off between selectivity to ethylene and propylene. It should be noted that the catalysts synthesized in our study are of higher Si/Al (≈ 200) and Ca/Al (> 9) ratio with nano-sized particles (≈ 150 nm).
The consecutive kinetics of the formation of higher olefin products in MTO reaction results in the higher production of
\( {\rm{C}}^{=}_{2}+{\rm{C}}^{=}_{3} \)
at the lower level of methanol conversion. Hence, lowering conversion could be used as a key parameter to adjust light olefins production.
Data in
Table 5
shows that the performance of Ca27-HZSM-5 and Ca54-HZSM-5 catalysts with respect to the yield of light olefins is nearly independent
of temperature very clearly. With decreasing temperature from 490 to 390 °C and at the methanol WHSV of 5.55 h−1, the yields of ethylene and propylene on Ca27-HZSM-5 remained nearly constant in the ranges of 0.10−0.11 and 0.13−0.14, respectively. The other catalysts showed an intense decline in their performance at 390 °C. Ca54-HZSM-5 catalyst presented
the lowest methanol conversion even at 490 °C and WHSV = 3.3 h−1 while other catalysts are much more active at this condition. Increasing Ca content of HZSM-5 at Ca/Al = 27 and 54 reduced methanol conversion but independent of temperature with overall enhanced yield of ethylene and propylene. It indicates a lower activation energy barrier of MTO reactions on the Ca LAS with respect to the BAS. Further increase of Ca content in HZSM-5 declines the MTO performance of HZSM-5. For example, Ca54-HZSM-5 with the highest Ca content presented the lowest methanol conversion (77%) at WHSV = 3.3 h−1 and
490 °C, whereas other catalysts resulted in the methanol
conversion > 93% at the same condition.
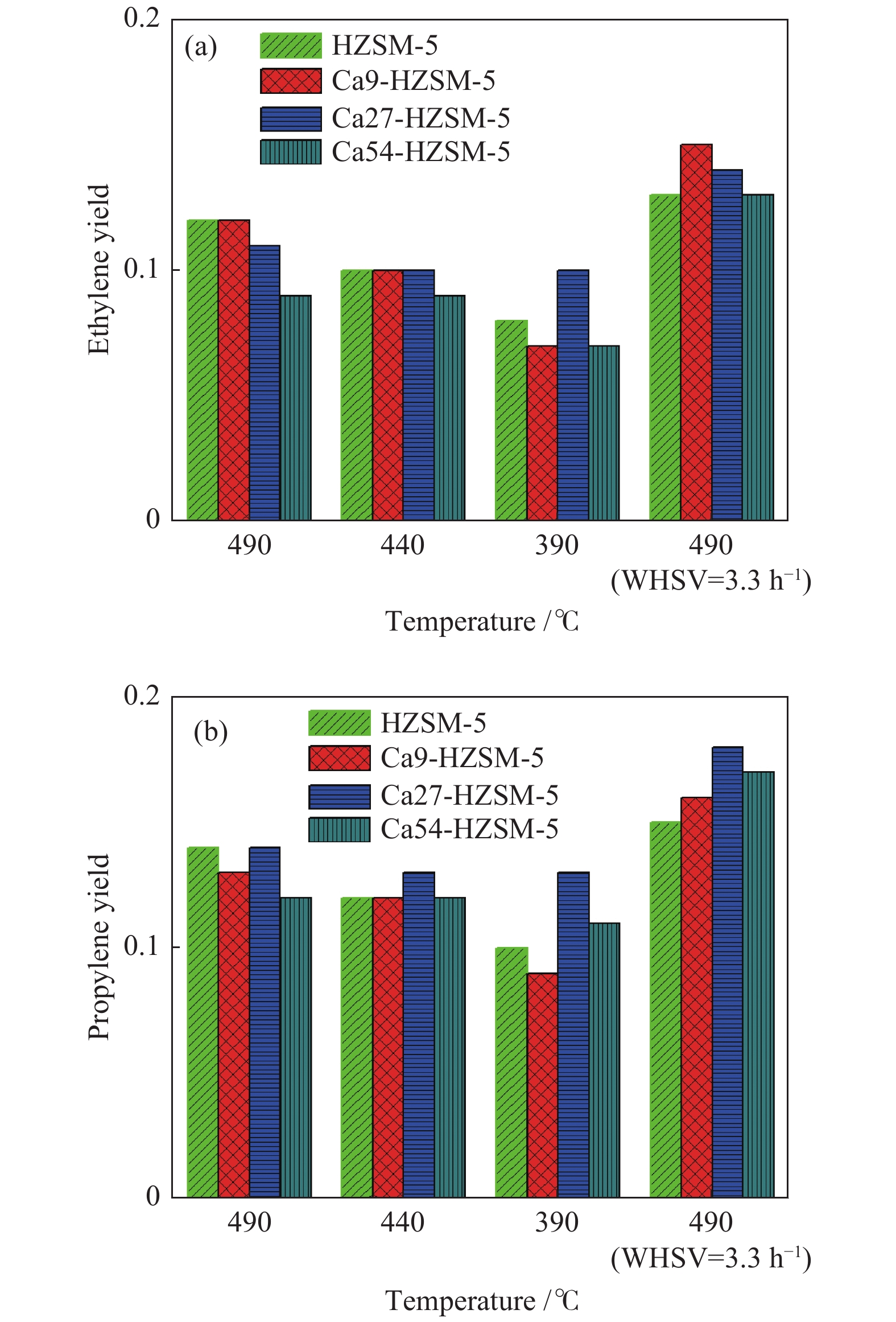
Fig. 5
Light olefin yield versus temperature for the prepared catalysts in the MTO conversion, Pressure = 100 kPa,
methanol WHSV = 8 and 4 h−1, Argon flow = 20 SCCM;
a) ethylene, b) propylene
In addition to the impact on the yield of products, it is noteworthy that Ca in the catalyst framework leads to a mitigating effect on the growth of carbon species. After the MTO performance test, the spent Ca-exchanged catalysts turned into gray color instead of black color for the parent HZSM-5 catalyst.
According to
Table 5
, the methanol conversions of catalysts are in the below order at 440 to 490 °C and WHSV ≈ 5.5 h−1:
HZSM-5 > Ca9-HZSM-5 > Ca27-HZSM-5 > Ca54-HZSM-5
However, at the lowest temperature, i.e. 390 °C and WHSV ≈ 5.5 h−1, the Ca27-HZSM-5 catalyst was the most active one. It could be attributed to its largest density of the strongest acid sites among Ca substituted catalysts, although these acid sites are weaker than HZSM-5 and the major portion are of Lewis acid type as confirmed by pyridine FT-IR data. The Ca LAS seems to drive MTO reactions with less activation energy.
The overall yields of
\( {\rm{C}}^{=}_{2}+{\rm{C}}^{=}_{3} \)
of catalysts are in the below order at 440 to 490 °C and WHSV = 5.5 h−1:
Ca27-HZSM-5 ≈ HZSM-5 > Ca9-HZSM-5 > Ca54-HZSM-5
Steric hindrance of Ca atoms in the Ca27-HZSM-5 zeolite structure with a decreasing effect on conversion, as well as the highest number of accessible Ca LAS (weaker than BAS on HZSM-5) among Ca incorporated zeolites, could lead to the acceptable activity and the high selectivity to light olefins. Ca54-HZSM-5 seems to be overloaded with Ca atoms and their steric hindrance may prevent acid sites to be available for MTO reactions.
It should be noted that despite the effect of Ca on the performance of HZSM-5 in the MTO reaction, the difference in the overall performance became less significant at the higher temperatures and the lower feed WHSV. In fact, the Ca effect on the MTO performance of HZSM-5 was pronounced at the higher WHSV. For instance, at methanol WHSV around
3.3 h−1 and 490 °C, Ca27-HZSM-5 converted 97% of methanol with selectivity to
\({\rm{C}}^{=}_{2}+{\rm{C}}^{=}_{3} = 87\%\)
and yield of
\({\rm{C}}^{=}_{2}+{\rm{C}}^{=}_{3}=32\%\)
compared to 76% and 28% over the parent HZSM-5 with 96% methanol conversion, respectively.
The peak areas of strongest acid sites (3th desorption
peak) in TPD profiles of the prepared catalysts follow the below order:
Ca27-HZSM-5 > Ca9-HZSM-5 > HZSM-5 ≈ Ca54-HZSM-5
As it was pointed above, the larger peak areas indicate the larger portion of accessible Ca LAS.
Based on the temperature of the peaks centerlines in NH3-TPD profiles presented in
Table 4
, the strength of acid sites corresponding to the 3th desorption peak of the prepared catalysts follows the below order:
HZSM-5 (575 °C) > Ca27-HZSM-5, Ca54-HZSM-
5 (518 °C) > Ca9-HZSM-5 (468 °C)
HZSM-5 contains the highest Brønsted acid sites whereas Ca27-HZSM-5 has the highest portion of accessible Ca LAS with less strength than BAS. It means that the type or strength of acid sites both influence the catalytic performance (yields of
\( {\rm{C}}^{=}_{2}+{\rm{C}}^{=}_{3} \)
). The ratio of different types of strong acid sites vary in the amount of heavy olefin products. Hence, there should be an optimum portion of types of acid sites for the maximum light olefin production.
Acid properties of the prepared catalysts obtained by NH3-TPD profiles implied that the lowest density of accessible strong acid sites (almost 100% portion of Ca LAS) in Ca54-HZSM-5 among the prepared catalysts resulted in the lowest average methanol conversion and the highest
\( {\rm{C}}^{=}_{2} \)
and
\( {\rm{C}}^{=}_{3} \)
selectivities, respectively. Ca27-HZSM-5 catalyst contained the largest portion of accessible LAS sites. The resolved band corresponding to the strong acid sites indicated a shift of center line to the lower temperatures (517 °C) with respect to HZSM-
5 (575 °C). It led to its larger selectivity to
\( {\rm{C}}^{=}_{2} \)
and
\( {\rm{C}}^{=}_{3} \)
than that of the parent HZSM-5 and the largest yield of
\( {\rm{C}}^{=}_{2}+{\rm{C}}^{=}_{3} \)
.
According to the TPD profiles and the result of MTO performance of the prepared catalysts, two properties of zeolite acidity, i.e. the strength (and their type) and the density of the different types, were used to explain the results. Less density of BAS in zeolites decreases the methanol conversion whereas it enhances the selectivity to light olefins in the MTO conversion. It should be noted that probable transition-state-shape-selectivity induced by Ca cations may also cooperate with the reduced acid strength of Ca LAS to enhance the selectivity of lighter olefins.
Ca9-HZSM-5 catalyst showed the smaller density of Ca LAS and lower average strength of acid sites than Ca27-HZSM-5. According to pyridine-IR data, BAS only in Ca9-HZSM-5 catalyst was weakened. In agreement, its average strength of acidity (3rd peak in TPD) was obtained as the lowest among the prepared catalysts. However, it provided more methanol conversion than Ca27-HZSM-5. It may be described by the steric hindrance of higher Ca content of the latter zeolite limiting species transport or product transition state formation in its pore structure. Species with a larger hydrodynamic diameter than ammonia faces more diffusion barrier in the pore structure of Ca27-HZSM-5 than that of Ca9-HZSM-5, leading to the decline of methanol conversion to the MTO products. Another issue is the percentage of BAS in Ca9-HZSM-5 (68%) which is larger than that of Ca27-HZSM-5 (22%) as calculated from their pyridine adsorbed IR absorption spectra. Bridged OH Brønsted acid sites, even with reduced strength in Ca9-HZSM-5, may be more active than Ca associated Lewis acid sites in the zeolites.
It is noteworthy that the higher content of Ca in Ca54-HZSM-5 zeolite imposes transport limitation on both nitrogen as surface probe and ammonia as the probe of acid sites, leading to less measured surface area and density of acid sites, respectively. Therefore, the lowest density of acid sites is available to methanol in the pore structure of Ca54-HZSM-5 which resulted in the lowest methanol conversion. In addition, the portion of Brønsted acid sites extensively reduces to 18%.
The influence of Ca incorporation on the acidic properties of HZSM-5 as the catalyst on MTO product selectivity and its deactivation has been reported in the literature
47
,
46
,
64
,
48
. It has been observed that less acidic strength suppresses aromatic cycle in the MTO reaction pathway producing less ethylene and deactivating aromatic species. The weakened acid sites could not further convert olefins produced by methylation/
cracking in the alkene cycle into aromatics. Yarulina et. al have carefully studied modification of acidity strength of HZSM-5 by Ca incorporation
48
. In summary, the transformation of Brønsted into Lewis acidity by Ca modification were confirmed and the improved life time and increased selectivity to propylene of Ca modified HZSM-5 was related to the decline of acid strength proved by NH3-TPD data. Reduction of acid sites density was not observed in the NH3-TPD spectra, despite revealing the transformation of Brønsted into Lewis acidity by Pyridine FTIR. The theoretically calculated OH frequency shift of CaOCaOH+ as proposed stable species in Ca-HZSM-5 indicated a weaker Brønsted acidity
48
.
The area of the third NH3 desorption peak in TPD spectra increased in Ca9-HZSM-5 and Ca27-HZSM-5, and decreased again at the highest Ca content in Ca54-HZSM-5. This decline of peak area may be explained by the possible steric hindrance effect of Ca atoms becoming important after a threshold content. It means Ca incorporation could result in the increase of LAS, however, the associated steric hindrance could inhibit the accessibility of acid sites. Diffusion limitation in Ca2+ incorporated HZSM-5 may be caused by its larger size than H+ and its substitution location in the pore structure. The covalent radius of Ca (1.73 Å) is more than 5 times of H (0.32 Å). This shows a larger distance of Ca from pore walls than H. Ca preferentially
exchanges with H at channel intersections
38
, where the acid sites favor aromatic cycle of MTO. However, it begins to exchange at the channels wall at higher content with clearly stronger negative effect on pore diffusion.
The strength of acid sites was declined by Ca incorporation in Ca9-HZSM-5 and then was enhanced again in Ca27-HZSM-5 and Ca54-HZSM-5 along with the intensified increase of peak areas. The decline in the strength of acid sites and conversion of Brønsted to Lewis acid sites with Ca incorporation has been observed and explained by other researchers, too
48
. However, the reason for the re-increase of strength of acid sites with Ca content could be inferred from pyridine IR data. The strength of BASs in Ca9-HZSM-5 was decreased and strengthened again in Ca27-HZSM-5 and Ca54-HZSM-5. However, the average strength of strong acid sites in the latter catalysts, is still less than those of the parent HZSM-5 due to the dominance portion of LASs. The LAS portion in two latter catalysts are nearly the same. Hence, the center of the third peak, related to the average strength of strong acid sites are the same in two latter catalysts.
2.7
MTO Activity versus time
The long- term performance tests were carried out for the duration of ca. 100 h on the HZSM-5 basis catalyst and the Ca modified catalyst with the best performance, i.e. Ca27-HZSM-5, to compare their MTO performances at 490 °C and methanol WHSV ≈ 9 h−1. The results of long term performance tests were displayed in
Figure 6
. In this experiment less amount of catalyst (= 0.1 g) was used, resulting in the lower methanol conversion than short time experiments. The HZSM-5 sample was regenerated at 650 °C for 5 h at static condition for use in the long term performance experiment. It was observed that this regeneration condition is not completely effective for the prepared catalyst and conversion was not recovered completely (57% instead of 79%). Furthermore, the selectivity to ethylene and propylene significantly was reduced over the regenerated catalyst which was compensated by the increased selectivity to
\( {\rm{C}}^{=}_{4} \)
and
\( {\rm{C}}^{=}_{5} \)
olefins. The same result was observed at several performance tests conducted on the regenerated catalysts prepared in this study.
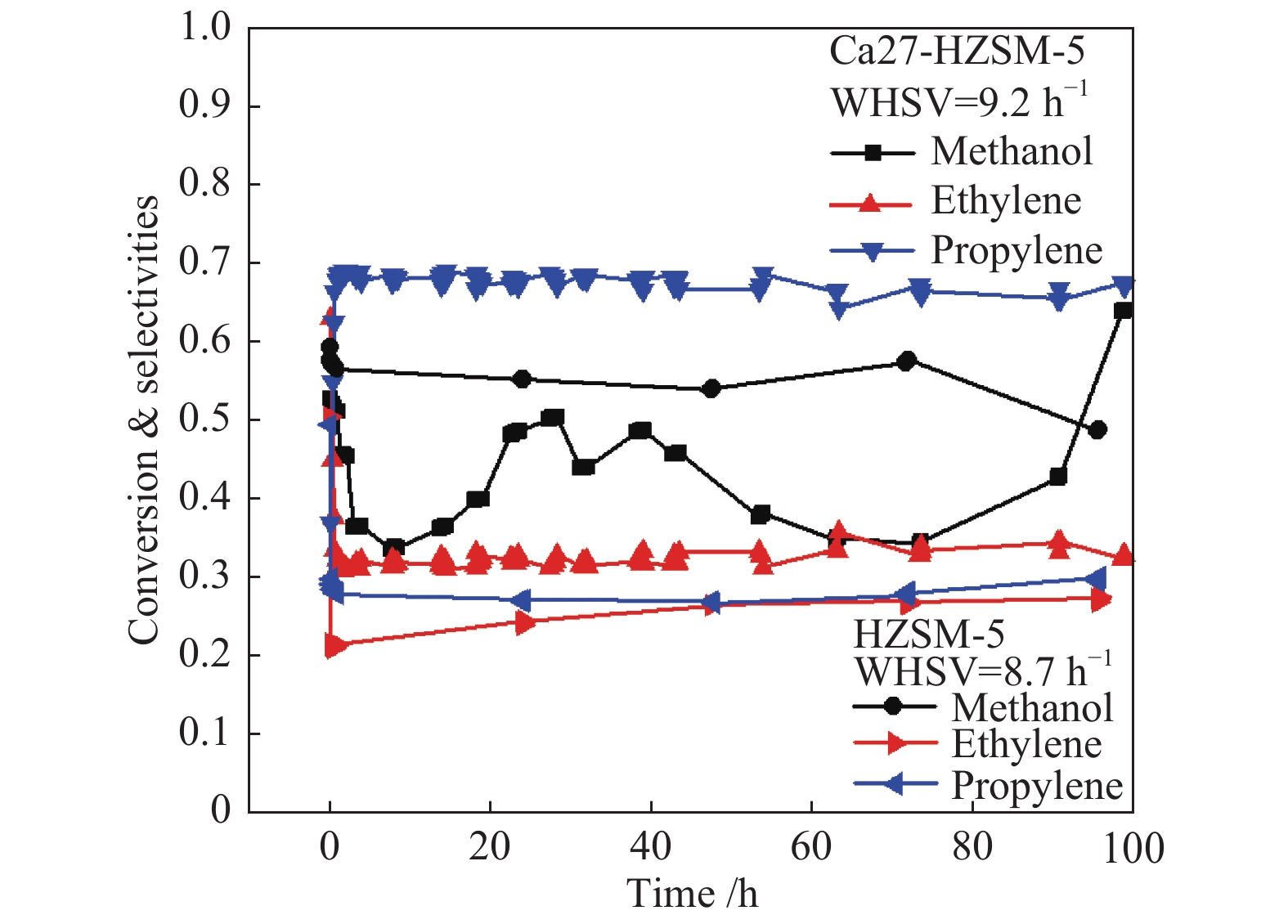
Fig. 6
methanol conversion and selectivity of olefins versus time for the prepared catalysts in the MTO conversion,
Pressure = 100 kPa, catalyst weight = 0.1 g, Argon
flow = 20 SCCM. Regenerated HZSM-5 was used
in this test which its initial conversion (57%) is
less than that of fresh sample (79%). The HZSM-5
catalyst in this test was regenerated at 650 °C for 5 h
With less initial activity of Ca27-HZSM-5 (53%) than HZSM-5 (59%), methanol conversion followed an oscillatory trend over both catalysts with different intensity. This oscillatory trend has also been observed in other similar studies on MTO conversion using Ca modified HZSM-5 in our group
49
.
Figure 6
shows that Ca27-HZSM-5 recovers its initial conversion in 24 h after which the second wave appears. During the second wave, the period of rising activity started at time on stream ≈ 70 h and methanol conversion reached to 64% at 99 h, higher than the initial value. The methanol conversion over HZSM-5 was recovered to 58% after about 70 h followed by a decline to 48% in the next 24 h. Considering selectivity of light olefin products with time on stream over both catalysts, it can be noticed that selectivities are more stable than methanol conversion.
These results determined a more thorough research is required for any conclusion on the long term MTO performance as a result of Ca incorporation to HZSM-5 zeolite. Although catalytic activity of HZSM-5 showed small fluctuations, it presented a more stable MTO activity than Ca27-HZSM-5. Ca27-HZSM-5 lost about 30% of its activity at 70 h, however, it became more active after 100 h. It is required to carry out an activity test of longer duration to determine stability preference. The oscillation of MTO activity of catalysts at the condition of nano size particles of catalysts and high methanol WHSV, may be resulted from the sequential formation and break down of carbonaceous species inside the porous structure of catalysts.
If the oscillatory feature of MTO activity of Ca27-HZSM-5 continues to longer times on stream, the deactivation could be slowed down as an advantageous feature.
2.8
Analysis of Coked Catalyst
2.8.1
FT-IR Results
FT-IR spectra of coked Ca27-HZSM-5-LT and Ca54-HZSM-5 catalysts were recorded in the range of 400−4000 cm−1, displayed in Figure S6 of the supplementary material file. Ca27-HZSM-5-LT denotes the coked Ca27-HZSM-5 catalyst after long term MTO activity test. The IR bands at 1580 cm−1 and 1650 cm−1 are assigned to stretching mode of aromatic C=C bonds in poly-aromatic hydrocarbons (PAH) or in coke (dienes)
65
-
67
. The band at 1580 cm−1 is not present in the FT-IR spectra of fresh catalysts. The carbon deposits containing PAH are called Coke-II. The band at 2930 cm−1 is most likely due to aliphatic −CH2 and −CH groups, and the band at 2960 cm−1 to −CH3 aliphatic
groups
66
and the band at 3050 cm−1 to stretching mode of C−H aromatic bonds
65
. The vibration mode at 1506 and 1414 cm−1 corresponds to CH2 and CH3 bending
65
. Weak band at 730 cm−1 in the FT-IR spectrum of Ca27-HZSM-5-LT is ascribed to bending vibration of mono and ortho di-substituted methyl aromatic C−H bond.
The strong band at 1729 cm−1 corresponds to the stretching mode of C=O bond, assumed as a sign of formation of oxygenated carbon deposit. It is observed in FTIR spectra of both coked samples, however, it is intensified in the Ca27-HZSM-5-LT. It can be deduced that the oxygenated coke, Coke-I, is formed at early times on stream and remains on the catalyst for a long period. The C=O band also exists on the FT-IR profile of fresh catalyst each.
2.8.2
Thermogravimetric Results
TG profiles of the used Ca27-HZSM-5-LT and Ca54-HZSM-5 catalysts, as shown in
Figure 7
, indicated
an intense effect of Ca incorporation on the amount of coke formed in the porous structure of the HZSM-5. The time on stream of methanol for HZSM-5 was ca.
5 h, while Ca27-HZSM-5-LT was catalyzing the MTO reaction for about 100 h.
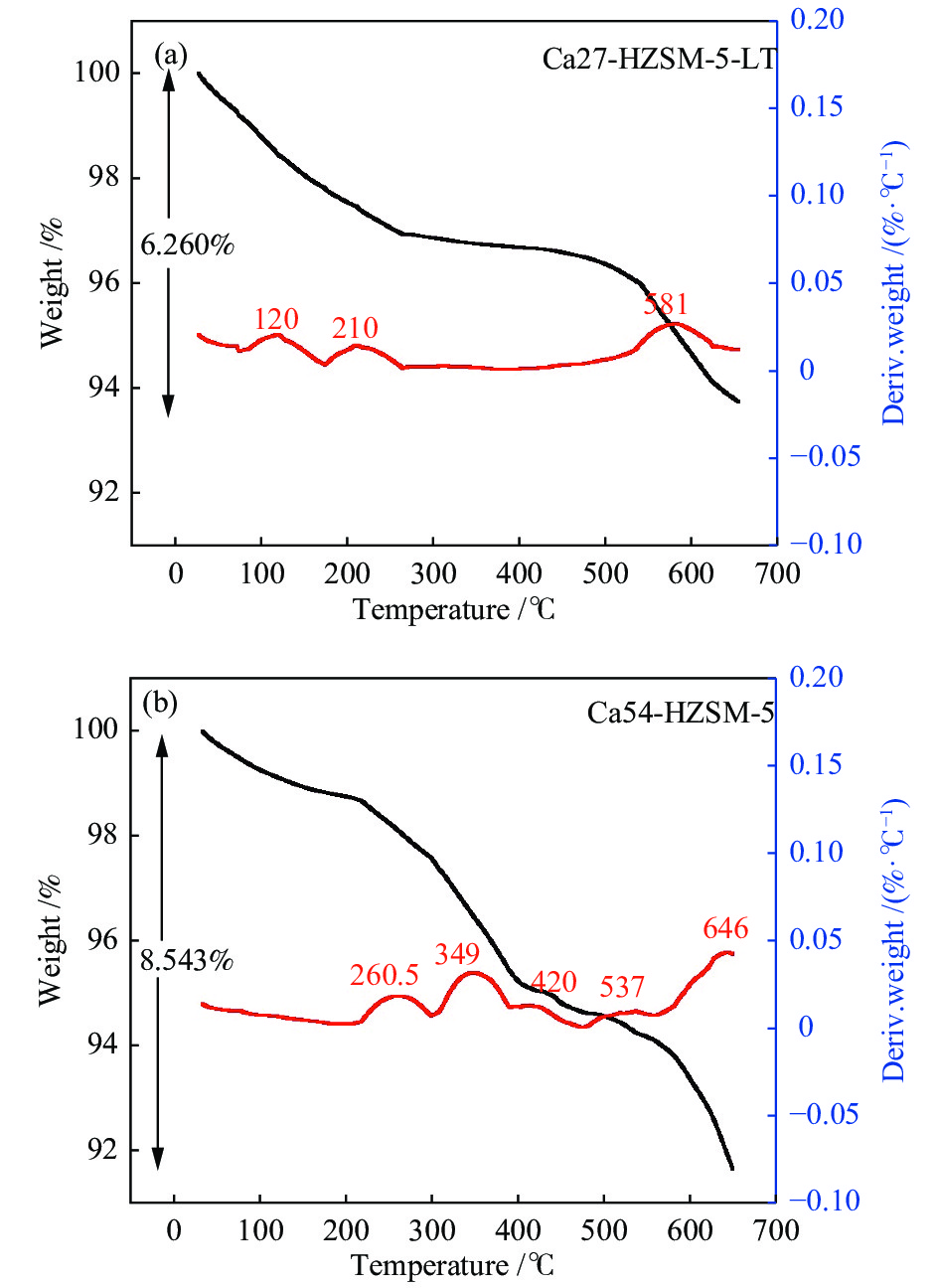
Fig. 7
Thermogravimetric and differential weight loss profiles of a) the spent Ca27-HZSM-5-LT, and
b) Ca54-HZSM-5 catalysts
According to the TG data, Ca27-HZSM-5-LT showed 6.3% weight loss after 100 h time on stream, whereas Ca54-HZSM-5 contained at least 7.5% carbonaceous deposits after 5 h interval. 2 % of weight loss in Ca27-HZSM-5-LT accounts for moisture dried at ca. 120 °C. Hence, Ca27-HZSM-5 encountered carbonaceous deposits of less than 4.3 wt.% which is well below coke content on Ca57-HZSM-5.
Carbon deposits on Ca54-HZSM-5 experienced a range of combustion temperatures from 420 to 646 °C. The descending end of TG profile of Ca54-HZSM-5 indicated weight loss could be continued over higher temperature than 650 °C.
First, this carbonaceous precipitate on both of the used catalysts are less than 10 wt.% which is an advantageous feature achieved by Ca incorporation, especially at fairly high space velocity used in the study. Another important result is that the amount of coke precipitation remained constant in the long period of reaction over Ca27-HZSM-5-LT as it was less than that of Ca54-HZSM-5 on which MTO reaction carried out for only 5 h. Therefore, the proper content of Ca in HZSM-5 not only mitigates the rate of coke formation but also stands for coke growth over long periods effectively.
Several bands can be seen in the derivative plot of TG profiles. Bands with maximum temperature less than 200 °C in DTG curve are attributed to adsorbed water and permanent gas molecules. Carbonaceous species over Ca27-HZSM-5-LT burnt at 210 and 581 °C
whereas DTG profile of Ca54-HZSM-5 indicated four different species burning at 349 to 646 °C. The higher combustion temperature indicates more condensed, higher graphitization and lower H/C ratio of carbon deposits
66
,
68
. It means that at the early stage of MTO, coke deposits are formed with different degrees of condensations over Ca54-HZSM-5. After a long time on stream the composition of coke deposits turns into the highest possible molecular weight over Ca27-HZSM-5-LT, i.e. progressively from the intermediate poly-alkylbenzenes to inactive and more condensed polycyclic aromatic structures
68
. In another term, oxygenated coke deposits, such as the species burnt at lower temperatures such as 210 °C over Ca27-HZSM-5 or 350 to 420 °C over Ca54-HZSM-5, called Coke-I, converts with methylation to polycyclic aromatics burnt at 536 to 646, called Coke-II, or the single type coke deposit over Ca27-HZSM-5-LT removed at 581 °C.
Coke-I as an oxygenated carbon species burnt at lower temperature deactivates HZSM-5 faster than the Coke-II as a poly aromatic species. It has been previously reported that two kinds of carbonaceous species can be formed inside pores of HZSM-5 in the MTO conversion, deactivating HZSM-5 by site blocking
68
. The conversion process of Coke-I to Coke-II with time on stream has been previously suggested by several research groups
68
,
66
.
It is believed that MTO reaction is driven by a hydrocarbon pool (HCP) mechanism based on a dual mechanism, Alkene-based route and aromatic-based route
38
,
69
. In the Alkene-based route heavier olefins are active HCP species, while poly-methyl-benzenes are the active HCP intermediates in the other
38
. MTO on the Ca containing HZSM-5 catalysts has been reported to proceed over the alkene cycle as the dominant route due the steric hindrance effect resulting from Ca ions exchanged with H+
48
,
38
. Our results indicated the formation of Coke-II which confirms the aromatic-based driving mechanism route is active, too.
The difference of composition of coke deposits formed over Ca27-HZSM-5-LT and Ca54-HZSM-5 is clear. The Coke-II over Ca54-HZSM-5 removed at about 646 °C is heavier than Ca27-HZSM-5-LT burnt at 581 °C. Hence, Ca content may interrelate with the composition of carbon deposit formed on the HZSM-5 in MTO reaction. Moreover, the amount of carbon deposits could be influenced by Ca incorporation in HZSM-5. Therefore, Ca incorporation can be employed to control both light olefin selectivity and lifetime in MTO conversion.
3
Conclusions
The methanol conversion and selectivity to light olefins for the parent and Ca modified HZSM-5 catalysts (9 ≤ Ca/Al ≤ 54) were measured. It was found that there is an optimum Ca content in HZSM-5 to obtain the best MTO performance. Ca27-HZSM-5 (Ca/Al = 27) showed the best catalytic performance with the yields of ethylene and propylene equal to 0.11 and 0.14 at 490 °C, respectively. Ca incorporation in HZSM-5 significantly decreased coke formation as calculated through TG analysis and deduced from the intensity of color change after reaction. Overloading of Ca in HZSM-5 led to formation of inaccessible LAS.
Ca modification of HZSM-5 improved the yield of ethylene and propylene and led to independency of yield of light olefins on temperature. It implies the decrease of energy barrier of MTO on Ca incorporated ZSM-5 in the studied range of temperature.
The performance of the evaluated catalysts in the MTO reaction were explained through deconvoluted FT-IR and NH3-TPD profiles based on the BAS/LAS ratio, steric hindrance and strength of acid sites. Ca27-HZSM-5 contained the largest number of accessible LAS. BAS include 78% of total acid sites in Ca27-HZSM-5. The effect of Ca content of HZSM-5 on the wavenumber of BAS vibration band, meaning their strength variation, was observed in FT-IR spectra of pyridine adsorbed zeolites. Ca incorporation weakens BAS at a threshold after which BAS strength is recovered again.
The catalyst stability experiment indicated that both oxygenated and polyaromatic carbon deposits were formed over the Ca incorporated HZSM-5 catalysts. Proper Ca content may lead to the formation of lighter aromatic carbon deposits.
The long-term MTO activity test showed an intense oscillatory behavior over Ca27-HZSM-5-LT and the higher activity than initial value after about 100 h.
Conflicts of interest
There are no conflicts of interest to declare.
Acknowledgement
Financial support of South Pars Gas Complex (S.P.G.C), Vice-Presidency of Research and Technology
is gratefully acknowledged.